OXYGEN TRANSMISSIBILITY
Corneal Oxygen: 2015
A look at what we know about corneal oxygen needs during contact lens wear and oxygen transmissibility through contact lenses.
By Barry A. Weissman, OD, PhD, FAAO (Dipl CCL), Dipl ABO
Optical correction of errors in human refraction is restricted to spectacles, contact lenses, and refractive surgery. Each form of optical correction has its advantages and disadvantages as well as its risks and benefits. For readers of this publication, contact lens wear is (arguably) the most elegant.
For more than a century, entrepreneur/inventor(s), scientists, and clinicians have labored to provide effective and safe contact lenses to optically correct conditions including myopia, hyperopia, astigmatism, and presbyopia. Improvements in contact lenses for therapeutic use (e.g., aphakia, keratoconus, orthokeratology, prosthesis, bandage, etc.) have followed in the wake of advances in safe and effective refractive contact lenses.
Contact Lens-Driven Complications
There is a long list of (mostly minor) complications that can occur with contact lens wear: epithelial staining and abrasion, edema and infiltrates of the epithelium and stroma, giant papillary conjunctivitis, neovascularization, and microbial infection, to name a few of the more prevalent.
Understanding the role that oxygen plays in normal metabolism, and recalling that the first contact lenses were made from non-oxygen-permeable glass and plastics, it is no surprise that many of the known contact lens complications have been identified as etiologically related to lack of sufficient oxygen supply reaching the anterior corneal surface through the tears (Goodlaw, 1946; Smelser and Ozanics, 1952; Kleinstein et al, 1981), either directly from the atmosphere (open eye) or indirectly from the lids’ palpebral conjunctiva (closed eye).
Acute hypoxia is now known to cause metabolic changes in the corneal epithelium, stromal acidosis, and corneal swelling secondary to suppression of endothelial hydration control function (Korb and Exford, 1968; Polse and Mandell, 1970; Zantos and Holden, 1977; Klyce, 1981; and others. Full list available at www.clspectrum.com/references.).
Clinically, chronic hypoxia leads to microcysts and decreased sensitivity, thickness and adhesion in the corneal epithelium, neovascularization and thinning in the stroma, and change in endothelial cell shape as well as function (Zantos and Holden, 1977; Millodot and O’Leary, 1980; Holden et al, 1985; Madigan et al, 1987; and others).
Critical Oxygen Tension
Polse and Mandell (1970) were the first to propose and identify a hypoxia metric, a “critical oxygen tension” (COT) for the anterior corneal surface, below which corneal metabolism becomes compromised. Atmospheric oxygen tension (at sea level) is 21% of 760 mmHg or about 155 to 160 mmHg (the remainder is mostly nitrogen, with a small percentage of water vapor and other gases). At higher elevations, where total atmospheric pressure falls, the percentage of oxygen in our atmosphere remains stable, but the pressure of oxygen lessens, equaling 21% of perhaps 600 mmHg (or 120 mmHg) on some tall mountains.
By use of goggles through which humidified gases of specified oxygen concentrations were passed over human corneas, the COT to preclude corneal swelling was first quantified at 11 to 19 mmHg (Polse and Mandell, 1970). Later research, however, increased this COT to closer to 100 mmHg (Mandell and Farrell, 1980; Holden et al, 1984; Papas, 1998; Weissman and Ye, 2006).
Other proposed physiological COT metrics include corneal touch sensitivity (at 60 mmHg) (Millodot and O’Leary, 1980), epithelial lactate production and mitosis rate (at 100 mmHg) (Hamano and Hori, 1983), and epithelial mitochondria redox state (at 75 mmHg) (Masters 1984).
While the sea-level atmospheric oxygen tension (in front of the tear layer of the open eye) is about 155 mmHg, the palpebral conjunctiva only produces an oxygen level of 50 to 60 mmHg. It is, therefore, obvious that there will be some level of hypoxia during any prolonged closed-eye experience (e.g., sleep). This, combined with changes in tear tonicity, results in mild corneal swelling (3% to 4%) in normal human eyes upon awakening (Mandell and Fatt, 1965). Therefore, even highly oxygen-permeable contact lenses, if worn during sleep, will result in corneal hypoxia.
While the corneal biophysicists (e.g., Claes Dohlman, MD, PhD; David Maurice, PhD; Irving Fatt, PhD; and their students and colleagues) spent the last century identifying and quantifying human corneal oxygen requirements (to preclude metabolic disruption, first clinically observed as corneal swelling/edema), material research advanced to produce contact lens materials of increased oxygen permeability (Gaylord, 1974; Tanaka, 1979).
The initial soft/hydrogel contact lens material—polyHEMA (Wichterle and Lim, 1960)—was, at first, believed to be quite permeable to water, nutrients, and oxygen (Morrison and Edelhauser, 1972; Fatt, 1989). However, this was soon disproved (Fatt and St. Helen, 1971). Compared to the old polymethylmethacrylate (PMMA, from which “hard” contact lenses were made), there was indeed an “oxygen advantage” to early soft contact lenses, but this advantage was minimal compared to the now recognized corneal requirement.
Higher-oxygen-permeable contact lens materials—first silicone acrylates, then fluorinated silicone acrylates in rigid materials, followed by silicone hydrogels in soft materials—have been developed over the last several decades.
Oxygen Transmissibility Theory
Dr. Fatt is principally credited with developing the theory and the practice of measuring contact lens oxygen parameters. He developed a polarographic method based on work performed by Japanese polymer chemists for the food packaging industry (Aiba et al, 1968; Fatt and St. Helen, 1971).
Oxygen permeability (termed Dk, in which D is the oxygen diffusion coefficient and k is oxygen solubility) is an intrinsic material property. Dk is directly dependant on a material’s oxygen permeable moieties (for both rigid and hydrogel plastics), usually silicone and/or water (Fatt, 1978; Refojo, 1984). Oxygen transmissibility is termed Dk/t, in which t is lens thickness (Fatt, 1978; Refojo, 1984). Both Dk and Dk/t are laboratory “in-vitro” measurements (Fatt and St. Helen, 1971; Fatt, 1978).
Dk is measured in units of x10-11 (cm2/sec) (ml O2/ml mmHg). Dk/t, in which t is in centimeters, is measured in x10-9 (cm/sec) (ml O2/ml mmHg). Benjamin et al (2006) proposed “Fatt Dk units” as alternate units for Dk to honor the late Professor Fatt for his lifetime contributions to this practical science, and this will be used below. Likewise, Dk/t is then measured in Fatt Dk/t units.
Dk/t measurement is complex. First, material thickness is variable, both between different lenses and from point to point on any optically powered lens. Some assume that contact lenses have uniform thickness (as contact lenses are usually quite thin compared to their areas). Optically powered contact lenses, however, have relatively uniform thickness only at low minus (about –0.75D) optical powers (Weissman, 1982).
Morris and Fatt (1977) suggested that the central thickness value was reasonable to use for contact lenses of optical powers up to ±1.50D. Later authors proposed mathematical techniques to calculate average thickness, especially for optical powers greater than ±1.50D (Fatt 1979; Brennan 1984; Weissman 1986). Both Holden and Mertz (1984) and Tomlinson and Bibby (1985) suggested that contact lens thickness could be averaged over a central 6mm diameter (rather than the entire contact lens diameter). Fatt and Neumann (1989) noted, however, that average thickness is not appropriate for evaluating contact lens Dk/t, arguing that the thickest lens area is most important because it is the Dk/t at that site that will produce the greatest hypoxic stress to the underlying corneal tissues.
As theory evolved, increased contact lens Dk values necessitated improved accuracy of polarographic Dk/t measurements; to achieve this, corrections for both the fluid boundary layer effects in the measurement cell (which lead to under-estimations of Dk and Dk/t) and the “edge effect” that results from the polarographic sensor collecting oxygen from an area greater than its own diameter (leading to over-estimations of Dk and Dk/t) were introduced (Fatt and Chaston, 1982; Fatt et al, 1987; Brennan et al, 1987; Fatt and Mueller, 1994).
Early soft contact lenses had values of about 4 Fatt Dk/t units (Fatt, 1989), which was soon recognized as physiologically inadequate. Many of today’s GP and soft lenses have Dk/t values of 100 to 150 Fatt Dk/t units.
What is the best criteria by which to judge corneal oxygen supply? As noted above, several authors used COT as a marker for oxygen adequacy (Polse and Mandell, 1970; Mandell and Farrell, 1980; Holden et al, 1984). Both Holden and Mertz (1984) and Harvitt and Bonanno (1999) proposed critical Dk/t values (ranging, for different functions, from 35 to 125 Fatt Dk/t units) below which corneal physiology might be compromised. However, Fatt (1996) noted that Dk/t was an in-vitro measurement and a “disappointment” as a measure of in vivo performance.
First, oxygen tension under a contact lens on an electric sensor is always zero at the steady-state; it is both undesirable and unlikely to reach zero under a contact lens on a living eye. Secondly, the flux of oxygen across a contact lens on a polarographic sensor tends toward infinity as its t decreases to zero—but the in-vivo limiting case (e.g., no lens on an eye) is an oxygen flux into the cornea sufficient to sustain its metabolism (about 5µl to 10µl O2/cm2 hr) (Hill and Fatt, 1964; Larke et al, 1981).
In vivo oxygen flux was considered a potentially useful metric when Dk/t values were low, but flux changes minimally with wear of contact lenses of about 100 Fatt Dk/t units (Brennan, 2005).
Brennan (2005) alternately proposed percentage of normal corneal oxygen consumption as another possible measure, but this has yet to be widely accepted. He developed an eight-chamber model that plots theoretical corneal/contact lens oxygen tension profiles; this is a revision of Fatt’s three-chamber model (Huang et al, 2004; Fatt and Ruben, 1999) with Harvitt and Bonanno (1999) corrections for altered oxygen consumption with corneal acidosis and newer closed-eye (60 mmHg [Efron and Carney, 1981; Holden and Sweeney, 1985]) and aqueous humor (24 mmHg [McLaren et al, 1998]) oxygen tensions.
It should be noted that the Brennan model includes several other theoretically important improvements, such as correcting for spurious oxygen consumption when the oxygen tension falls to zero within the cornea and incorporating several more modern thickness estimates.
Figure 1 shows the results of calculations for predicting tear oxygen tension (pO2) under soft contact lenses (with minimal underlying tear layers) for sample values of Dk/t ranging from 10 to 150 Fatt Dk/t units using the original Fatt et al (Fatt and Bieber, 1968; Fatt et al, 1974; Fatt and Weissman, 1992; Fatt and Ruben, 1993)/Huang et al (2004) model, Brennan’s (2005) more complex model, and the Weissman and Ye (2006) version of the Fatt model. Table 2 shows the boundary conditions used to develop Figure 1. There are minimal differences in predicted tear layer pO2 with open-eye conditions, and only slight differences under closed-eye conditions, for all three models.
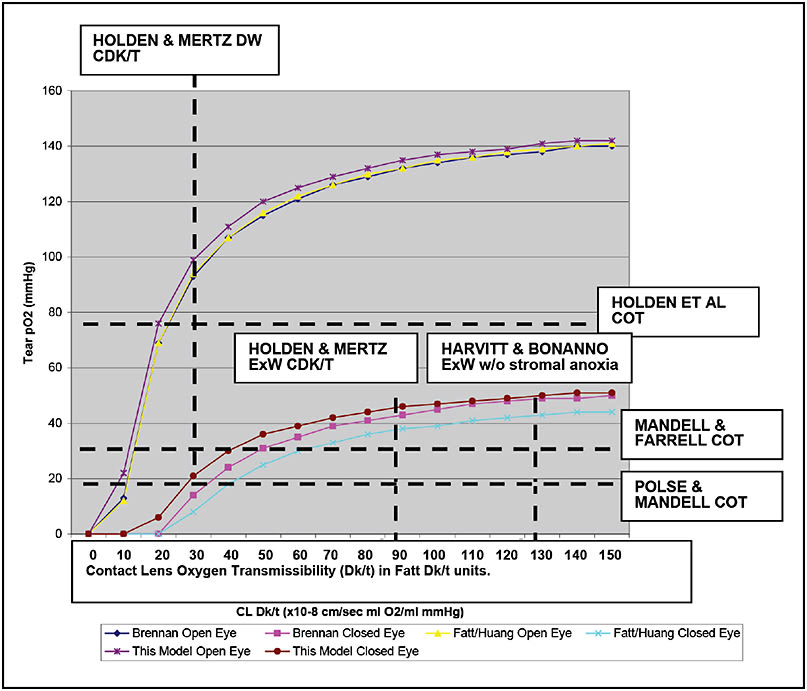
Figure 1. Tear layer oxygen tension (in mmHg) predicted by the original Fatt/Huang model, the Brennan model, and the current models for various contact lenses from 10 to 150 Fatt Dk/t units (or x 10-9 cm/sec ml/ml mmHg) is graphed. The upper curves are all open-eye predictions, and the lower curves are all closed-eye predictions. Benchmarks of the Polse and Mandell (1970), Mandell and Farrell (1980), and Holden et al (1984) critical corneal oxygen tensions (COTs) (11 to 19, 23, and 75 mmHg, respectively) and the Holden and Mertz (1984) and Harvitt and Bonanno (1999)* suggested critical Dk/t values (24, 87, and 125 Fatt Dk/t units, respectively) (dashed lines) are shown for historical reasons. (Figure reprinted from Weissman and Ye, CLAE 2006, with permission.)
* This particular value has been supported by the later work of both Papas (1998) and Sweeney et al (2000), but contested by Brennan and Efron (1987).
tcor (microns) | Q (µl O2/cm2h) | P1closed (mmHg) | P1open (mmHg) | Paq (mmHg) | Dkcor (Fatt Dk units) | |
---|---|---|---|---|---|---|
Fatt and Ruben (1993) | 500 | 9.54 | 55 | 155 | 55 | 24.7 |
Harvitt and Bonanno (1999) | 495 | Various (by layer) | 61.4 | 155 | 24 | Various (by layer) |
Huang et al (2004) | 560 | 9.54 | 55 | 155 | 55 | 24.7 |
Brennan (2005) | 532 | Various (by layer) | 61.5 | 155 | 24 | Various (by layer) |
Weissman & Ye (2006) | 540 | 9.7 | 60 | 155 | 30 | 24.7 |
tcor = corneal thickness
Q = corneal oxygen consumption P1closed = closed eyelid oxygen tension P1open = open eye oxygen tension Paq = aqueous humor oxygen tension Dkcor = corneal oxygen permeability |
Note that predicted tear pO2 initially increases rapidly with increasing Dk/t (regardless of open- or closed-eye conditions) and approaches an asymptote after a Dk/t value of about 70 to 100 Fatt Dk/t units. Tear pO2 eventually stabilizes at about 150 mmHg under open-eye conditions and at about 50 to 60 mmHg under closed-eye conditions. Of interest, these are the expected boundary conditions without any lens on the eye.
Figure 1 shows various previously proposed “benchmarks” for critical tear pO2 and contact lens Dk/t for comparison. There is no clear-cut boundary between what should be physiologically questionable versus what should be tolerable. From this analysis, maintaining an open-eye tear pO2 of at least 100 mmHg would likely be physiologically tolerable for most corneas, which translates to critical Dk/t values of 70 to 100 Fatt units.
However, it is important to note that tear layers of any substantial thickness between or under contact lenses would decrease the system Dk/t (by increasing resistance by one or two additional layers of their own Dk/t values). Fatt (1977) suggested that piggyback contact lenses could be considered “resistance in series,” as this contact lens system results in two layers of oxygen resistance. Weissman and Ye (2006) elaborated this model.
Any tear exchange (such as occurs with GP lenses) would increase the tear pO2 at the corneal surface. The concept of tear exchange with GP lenses was thoroughly explored by Fatt and Lin (1976, 1977) and Fatt and Liu (1984) but has not yet been applied to the slightly different situations of GP scleral and hybrid contact lenses.
Both hybrid and GP scleral contact lenses are very large-overall-diameter (15mm to 24mm) lenses that can trap substantial tear reservoirs over the corneal surface. The entrapped fluid layer and contact lens should also act as resistance in series. Jaynes et al (2015) and Lee et al (2015) have considered these situations, respectively.
To fully define the anterior corneal oxygen status when such lenses are worn, however, additional questions must be answered, such as whether or not these tear layers are stagnant or if they mix, and whether tear exchange occurs during wear of these lenses—and if so, how much tear exchange?
Modern Contact Lenses
To put the above discussion into some perspective, many current silicone hydrogel soft contact lenses have Dk values in the 100 Fatt unit (or above) range. Considering that these will be made in reasonable thicknesses for the vast majority of prescriptions, it is unlikely that clinicians following patients who use these lenses solely for daily wear will ever note any hypoxic consequences (e.g., stromal swelling, corneal neovascularization, epithelial microcysts, limbal injection, etc.).
Similarly, GP lenses made of materials with similar Dk values (approaching or exceeding 100 Fatt units) should achieve similar or even enhanced physiological success (given the likelihood of reasonable tear exchange).
Use of contact lenses made from plastics of decreased Dk and/or of very thick design, however, may still produce corneal hypoxia. Similarly, extended wear using any contact lens may also result in corneal hypoxia and its complications.
Moreover, as noted above, piggyback lenses and both scleral GP and hybrid contact lenses, which entrap substantial tear layers, then act, by resistance in series, to decrease the in vivo oxygen permeability of lens + tear systems. The resultant pO2 can approach the low-oxygen environments generated by both the non-oxygen-permeable rigid and the low-water-content and thick hydrogel lenses of the past (Weissman and Ye, 2006; Michaud et al, 2012; Jaynes et al, 2015).
Thus, clinicians who care for such patients should consider fitting to achieve modest rather than generous tear layers and optimizing, if possible, tear exchange. In addition, such patients should undergo closer professional supervision, and both patients and practitioners should remain vigilant for signs of contact lens-induced anterior ocular hypoxia. CLS
Acknowledgement: As always, Dr. Irv Fatt.
For references, please visit www.clspectrum.com/references and click on document #240.
![]() | Dr. Weissman is a professor of Optometry, Southern California College of Optometry at Marshall B. Ketchum University in Fullerton, CA, and emeritus professor of Ophthalmology, Jules Stein Eye Institute, David Geffen School of Medicine at UCLA, Los Angeles. He is a Diplomate in the American Academy of Optometry’s Cornea, Contact Lenses and Refractive Technologies section. He also is a Diplomate of the American Board of Optometry. Dr. Weissman has received research funding from SynergEyes. |